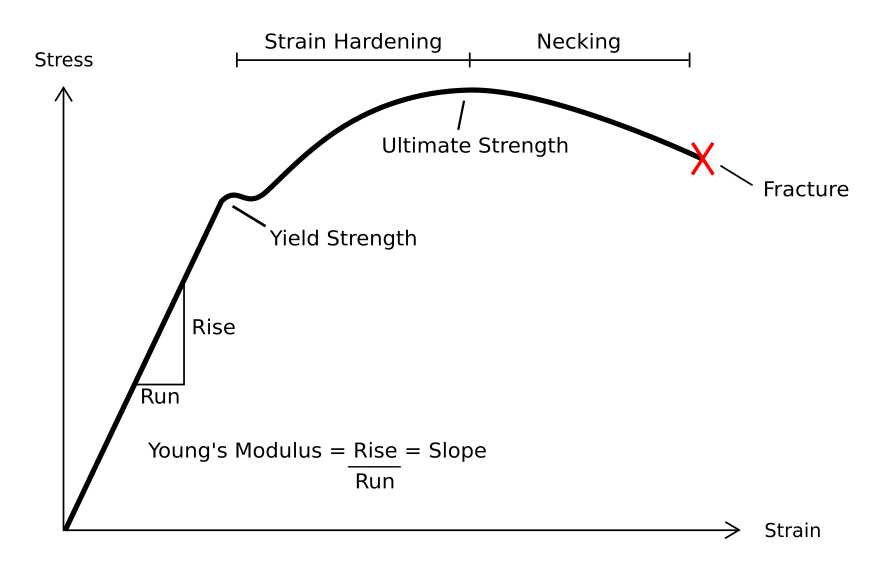
Have you ever wondered how materials are able to withstand extreme pressures and forces? The answer lies in the stress-strain curve, a valuable tool that helps us understand the resilience of various substances. By analyzing this curve, scientists and engineers are able to find the breaking point of a material and determine its ability to withstand stress.
The stress-strain curve is a graphical representation of the relationship between stress and strain in a material. Stress refers to the force applied to an object while strain measures the deformation or change in shape that occurs as a result of that force. By plotting stress along the x-axis and strain along the y-axis, we can visualize how a material responds to external forces.
Resilience is a key factor in determining the strength of a material. It is the ability of a substance to absorb energy and deform under stress without permanently changing its shape or structure. By analyzing the stress-strain curve, scientists can find the point at which a material begins to deform and determine its ultimate strength.
Unlocking the secrets of the stress-strain curve allows us to understand the limits of different materials and design structures that can withstand extreme conditions. By studying the curve, engineers can identify the optimal combination of materials for a specific application, ensuring that the final product is both strong and durable. So next time you encounter a stress-strain curve, take a moment to appreciate the wealth of information it provides and the incredible resilience it represents.
Understanding the Stress-Strain Curve
The stress-strain curve is a fundamental concept in materials science and engineering. It provides valuable insight into the behavior of materials under different levels of stress and strain. By analyzing this curve, researchers can gain a deeper understanding of the resilience and strength of a material.
The curve is obtained through experimental testing, where a material is subjected to increasing levels of stress until it reaches its breaking point. During this process, the material undergoes deformation, or strain, which is measured and plotted against the applied stress.
From the stress-strain curve, researchers can find several key points that characterize a material’s behavior. The elastic region of the curve represents the range of stress and strain where the material behaves elastically, meaning it can return to its original shape after deformation. This region is important for understanding a material’s resilience and ability to withstand external forces.
Beyond the elastic region, the curve enters the plastic region, where the material undergoes permanent deformation. This is where the material starts to exhibit plasticity, and its ability to return to its original shape decreases. The stress at which this transition occurs is known as the yield strength.
The curve continues to rise until it reaches the ultimate tensile strength, which is the maximum stress the material can withstand before breaking. This point represents the material’s strength under extreme conditions. Finally, the curve drops sharply after the breaking point, indicating the material’s failure.
Understanding the stress-strain curve is crucial for engineers and scientists in designing and analyzing structures and materials. By studying this curve, they can determine a material’s mechanical properties, such as its stiffness, toughness, and ductility. This knowledge allows them to make informed decisions about material selection and design optimization.
In conclusion, the stress-strain curve provides valuable insights into the behavior of materials under stress and strain. By analyzing this curve, researchers can understand a material’s resilience, find its yield strength, and determine its ultimate tensile strength. This knowledge is essential for designing and optimizing materials for various applications.
Exploring the Elastic Region
When studying the stress-strain curve, it is crucial to understand the concept of the elastic region. This region represents the range of stress and strain values in which a material can deform and then return to its original shape once the stress is removed.
The elastic region is characterized by a linear relationship between stress and strain, known as Hooke’s Law. According to Hooke’s Law, the stress is directly proportional to the strain, allowing us to calculate the modulus of elasticity, which is a measure of a material’s stiffness.
To explore the elastic region, we can analyze the initial part of the stress-strain curve. This section shows how a material responds to small amounts of stress, and it provides valuable information about its elastic properties.
By plotting stress against strain, we can determine the slope of the curve in the elastic region, which represents the material’s modulus of elasticity. This slope indicates how much stress a material can withstand before it starts to deform permanently.
Understanding the behavior of materials in the elastic region is essential for designing structures that can withstand various loads and stresses. By finding the elastic limit, engineers can ensure that materials used in construction projects have the necessary resilience to endure the expected stress and strain without permanent deformation or failure.
Investigating the Plastic Region
In the study of resilience, one of the key areas of focus is the plastic region of the stress-strain curve. This region represents the ability of a material to deform under stress without undergoing permanent damage or failure. Understanding how materials behave in this region is crucial for designing resilient structures and products.
To investigate the plastic region, researchers apply various levels of stress to a material and measure the resulting strain. By analyzing the relationship between stress and strain, scientists can determine the material’s ability to withstand deformation without breaking. This information is essential for engineers to design materials that can withstand the demands of real-world applications.
Researchers use a variety of techniques to study the plastic region, including tensile testing, where a material is subjected to tension until it reaches its breaking point. During this test, scientists can observe how the material deforms and measure the corresponding stress and strain values. They can also analyze the microstructure of the material to understand how it changes during deformation.
By studying the plastic region, researchers can find ways to improve the resilience of materials. They can identify the factors that contribute to the material’s ability to withstand stress and develop strategies to enhance these qualities. This knowledge can lead to the development of stronger, more durable materials that can withstand the challenges of various applications, from construction to aerospace.
Overall, investigating the plastic region is crucial for understanding the resilience of materials. By analyzing how materials behave under stress and strain, researchers can uncover the secrets of the stress-strain curve and find ways to enhance the resilience of various materials.
Factors Affecting the Stress-Strain Curve
The stress-strain curve is a graphical representation that shows the relationship between stress and strain in a material. It is an important tool for understanding the mechanical properties of materials and their resilience. Several factors can affect the shape and behavior of the stress-strain curve, including:
- Material composition: Different materials have different stress-strain behaviors due to variations in their atomic and molecular structures. For example, metals generally exhibit a linear elastic region followed by a plastic deformation region, while polymers may have more complex stress-strain curves.
- Temperature: The temperature at which a material is tested can significantly influence its stress-strain behavior. High temperatures can cause materials to soften and become more ductile, resulting in a different stress-strain curve compared to testing at room temperature.
- Strain rate: The rate at which strain is applied to a material can affect its stress-strain curve. Higher strain rates typically result in higher stresses and shorter plastic deformation regions. This is because the material has less time to accommodate the applied strain.
- Microstructure: The microstructure of a material, including factors such as grain size and crystal defects, can affect its stress-strain behavior. Materials with smaller grain sizes tend to have higher strengths and more pronounced strain hardening, leading to different stress-strain curves.
- External conditions: Factors such as humidity, pressure, and the presence of corrosive substances can also influence the stress-strain curve. These external conditions can cause changes in the material’s structure and chemical properties, affecting its mechanical behavior.
Understanding the factors that affect the stress-strain curve is essential for designing materials with desired mechanical properties and predicting their performance under different loading conditions. By studying these factors, researchers can find ways to improve the resilience and strength of materials.
The Role of Material Properties
The stress-strain curve is a valuable tool for understanding the resilience of materials. By analyzing this curve, researchers can find important information about the material’s ability to withstand stress and strain.
Stress is the force applied to a material, while strain is the resulting deformation or change in shape. The stress-strain curve shows how a material responds to increasing levels of stress, allowing researchers to determine its resilience.
Material properties, such as elasticity and plasticity, play a crucial role in shaping the stress-strain curve. Elastic materials can deform under stress but return to their original shape once the stress is removed. On the other hand, plastic materials undergo permanent deformation and do not return to their original shape.
By studying the stress-strain curve, researchers can identify the yield point, which is the stress level at which permanent deformation starts to occur. This information is valuable in determining the material’s strength and its ability to withstand stress without undergoing plastic deformation.
Understanding material properties is essential for engineers and scientists working in various fields. By analyzing the stress-strain curve, they can find insights into the behavior of different materials and make informed decisions about their use in different applications.
In conclusion, the role of material properties in the stress-strain curve is crucial for understanding the resilience of materials. By analyzing this curve, researchers can find valuable information about a material’s ability to withstand stress and strain, providing insights into its strength and durability.
Influence of Temperature on Resilience
The temperature at which a material is subjected to can have a significant influence on its resilience. Resilience is the ability of a material to absorb energy and deform without undergoing permanent deformation or failure. It is an important property in determining the material’s ability to withstand stress and strain.
When a material is exposed to different temperatures, its stress-strain curve shifts. At higher temperatures, the material tends to have a lower resilience. This is because the increased temperature causes the atoms or molecules in the material to vibrate more rapidly, leading to easier deformation and reduced ability to absorb energy.
On the other hand, at lower temperatures, the material tends to have a higher resilience. The decreased temperature slows down the atomic or molecular vibrations, making it more difficult for the material to deform. As a result, it can absorb more energy before reaching its breaking point.
Understanding the influence of temperature on resilience is crucial in various industries. For example, in the aerospace industry, materials used for aircraft components need to maintain their resilience even at extreme temperatures. Similarly, in the automotive industry, components subjected to high temperatures, such as engine parts, need to have high resilience to withstand the stress and strain.
Researchers and engineers study the temperature-resilience relationship to develop materials with desired properties for specific applications. By manipulating the temperature conditions during the manufacturing process, they can optimize the resilience of the material and ensure its performance under different operating conditions.
- Temperature can significantly affect the resilience of a material.
- Higher temperatures generally result in lower resilience.
- Lower temperatures generally result in higher resilience.
- Understanding temperature-resilience relationship is crucial in various industries.
- Researchers and engineers study this relationship to develop materials with desired properties.
Effect of Strain Rate on Stress-Strain Behavior
Stress and strain are two fundamental concepts in the study of materials and their mechanical behavior. Stress is a measure of the internal forces within a material, while strain is a measure of the deformation that occurs in response to those forces.
When a material is subjected to an external force, it deforms and experiences stress. The stress-strain behavior of a material can be characterized by plotting the stress and strain values on a graph, resulting in a stress-strain curve.
Resilience, or the ability of a material to absorb energy without permanent deformation, is an important property in many applications. Understanding the stress-strain behavior of a material can help us find ways to improve its resilience.
One factor that can affect the stress-strain behavior of a material is the strain rate, or the rate at which the material is deformed. The strain rate can have a significant impact on the mechanical properties of a material.
At high strain rates, materials tend to exhibit higher strengths and lower ductility. This is because the rate at which the material is deformed does not allow enough time for the atoms and molecules to rearrange and accommodate the applied stress. As a result, the material becomes stiffer and less able to absorb energy without permanent deformation.
On the other hand, at low strain rates, materials tend to exhibit lower strengths and higher ductility. The slower deformation rate allows more time for the atoms and molecules to rearrange and accommodate the applied stress. This leads to a more plastic deformation behavior, where the material is able to absorb more energy without permanent deformation.
Understanding how strain rate affects the stress-strain behavior of a material is crucial in many engineering applications. By studying the effect of strain rate, we can find ways to optimize the mechanical properties of materials and improve their resilience in various scenarios.
Applications of Stress-Strain Curve
The stress-strain curve is a valuable tool in understanding the behavior of materials under different levels of stress and strain. It provides valuable insights into the mechanical properties of materials and is used in various applications.
One important application of the stress-strain curve is in the field of material testing. Engineers and scientists use this curve to determine the mechanical properties of a material, such as its yield strength, ultimate tensile strength, and modulus of elasticity. By subjecting a material to different levels of stress and measuring the resulting strain, researchers can find out how the material behaves under different conditions.
Another application of the stress-strain curve is in the design and analysis of structures. By knowing the stress-strain behavior of different materials, engineers can determine the appropriate material to use in a particular application. They can also find out how much stress a structure can withstand before it starts to deform or fail. This information is crucial in ensuring the safety and reliability of structures.
The stress-strain curve is also used in the field of material selection. By comparing the stress-strain curves of different materials, engineers can find the most suitable material for a specific application. For example, if a material needs to withstand high levels of stress without deforming, engineers can look for materials with a high yield strength and ultimate tensile strength.
Furthermore, the stress-strain curve is used in the study of material fatigue and failure. By subjecting materials to cyclic loading and measuring the resulting stress-strain response, researchers can determine how long a material can withstand repeated loading before it fails. This information is crucial in designing materials and components that are resistant to fatigue and failure.
In conclusion, the stress-strain curve has numerous applications in various fields. It is a valuable tool for understanding the mechanical properties of materials, designing structures, selecting materials, and studying material fatigue and failure. By using the stress-strain curve, engineers and scientists can find out how materials behave under different levels of stress and strain, and make informed decisions in their respective fields.
Engineering Structural Design
In order to design resilient structures, engineers need to understand how materials respond to stress and strain. By studying the stress-strain curve, engineers can find the maximum stress a material can withstand before it starts to deform. This information is crucial for determining the structural integrity of a design.
When a material is subjected to stress, it undergoes strain, which is the measure of deformation. By analyzing the stress-strain curve, engineers can determine how much strain a material can endure before it reaches its breaking point. This knowledge allows engineers to design structures that can withstand the expected loads and forces.
Resilience, in the context of engineering structural design, refers to the ability of a material or structure to absorb and dissipate energy without permanent deformation. By understanding the stress-strain curve, engineers can find the point at which a material becomes permanently deformed, allowing them to design structures that can withstand and recover from stress.
By studying the stress-strain curve, engineers can also determine the elastic limit of a material. The elastic limit is the point at which a material can no longer return to its original shape after being subjected to stress. This information is crucial for designing structures that can withstand repeated loading and unloading cycles without permanent deformation.
In conclusion, engineering structural design relies on a thorough understanding of the stress-strain curve. By analyzing this curve, engineers can find the maximum stress a material can withstand, the amount of strain it can endure, and its ability to recover from stress. This knowledge is essential for designing resilient structures that can withstand the demands of their environment.
Mechanical Testing and Quality Control
In order to understand the resilience of materials, mechanical testing is conducted to find out how materials behave under stress and strain. Quality control measures ensure that the materials used in various applications meet the required standards.
Through mechanical testing, engineers and scientists can determine the stress-strain relationship of a material. This relationship helps in understanding how a material deforms when subjected to external forces. By applying controlled loads to a material and measuring the resulting strain, researchers can accurately determine the material’s mechanical properties.
Quality control plays a crucial role in ensuring that materials meet the desired specifications and perform as expected. By conducting mechanical tests, manufacturers can verify the strength, durability, and resilience of their products. These tests help identify any weaknesses or defects in the material, allowing manufacturers to make necessary improvements.
Mechanical testing also helps in understanding the behavior of materials under different conditions, such as temperature and humidity. By subjecting materials to different environmental factors, researchers can assess their performance and make necessary adjustments to ensure optimal functionality.
Overall, mechanical testing and quality control are essential in the discovery and development of resilient materials. By understanding how materials behave under stress and strain, manufacturers can ensure the production of high-quality and reliable products.

I am Patrina de Silva, a psychologist and mental health blogger in Sri Lanka. After obtaining psychology degrees from the University of Colombo and Monash University, I returned home to work as a counselor while also starting the popular blog “Pressy but Happy” to provide advice on psychological issues. Over the past decade, my empathetic articles have made my blog a leading mental health resource in the country. In addition to writing, I maintain a private therapy practice, frequently volunteer counseling time, and conduct seminars, driven by my passion for destigmatizing mental illness and educating the public on the mind-body connection. I strive to be an influential voice in my field through my compassionate approach.